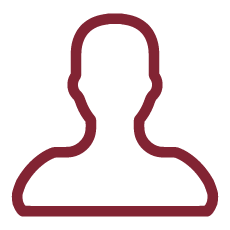
The quantum network, an infrastructure that supports intrinsically safe communication and connects quantum computers, is arguably the vision from the second quantum revolution which is closest to realization. The accomplishment of this enterprise requires quantum memory devices and on-demand single photon sources. These building blocks constitute the so-called quantum node. A quantum memory should be able to faithfully store flying qubits and release them on-demand, while a single photon source should emit on-demand single photons and, potentially, entangled photon pairs. To build quantum nodes, an approach which combines different quantum systems is likely to be the most rewarding. Semiconductor quantum dots, also dubbed artificial atoms, are emerging as near optimal sources of non-classical light, since they can emit single and polarization-entangled photon pairs with very high efficiency and at high repetition rate. On the other hand, alkali vapor cells have gained a lot of attention in the last years due to their capability of storing qubits even at room temperature without the need of sophisticated techniques. Moreover, it has been shown that they can also work as delay lines and spectral filters for flying qubits. Despite pioneering experiments in this field, a detailed description focused on the interconnection between these two physical systems is still lacking in the literature. In this project, a systematic study of this hybrid artificial-natural atomic system will be performed. GaAs quantum dots grown by droplet etching and integrated on a strain-tunable device will be used as sources of single and entangled photons in a micro-photoluminescence setup. In parallel, a 87Rb vapor cell will be investigated as a prospective quantum memory. Since our group's knowledge regarding the quantum dot topic is well established, emphasis will be devoted to optically characterize the vapor cell and test its suitability as quantum interconnect for quantum dot photons.
The successful development of a quantum network depends on the implementation of several building blocks. Among them, quantum memories that are suitable to store and retrieve single and entangled photons from quantum emitters are probably still far from a definitive realization. To date, several physical platforms for quantum memories are under investigation, ranging from cold atoms [1], electron spins in QD [2], and alkali vapor cells [3]. Alkali vapor cells are particularly attractive, as they do not require cryogenic temperatures or advanced laser cooling techniques---challenges that hinder large scale applications. However, the proper functioning of AC quantum memories has been demonstrated only for qubits generated by laser pulses probabilistically attenuated at the single photon level [4], whereas their operation with true single photon source has still to be demonstrated. Contrarily, on the source side an
optimal candidate has probably been found. In fact, it has been recently shown that semiconductor QDs can generate single and entangled photons on demand, with high efficiency, indistinguishability and purity----a set of properties not matched by any other
source of non-classical light [5]. In order to make QDs an attractive source for quantum networks, a matching quantum memory has to be developed. While seminal works on the hybrid artificial-natural atomic interface have been recently performed [6], the field is still in its infancy. Researchers all around the world are struggling with two main challenges: the first one consists in matching the energy of QD emission lines with those of alkali vapors. The second one is the matching of the bandwidth of QD photons (a few GHz) with the intrinsic bandwidth of the alkali atomic resonances (tens of MHz). Several approaches have been developed to tackle these challenges, but a full study of the QD-AC hybrid system is still lacking.
Within the above-described scenario, our project aims to make a step toward the development of QD-AC atomic interfaces. We will investigate how 87Rb AC interacts with single or entangled photons emitted by GaAs QDs and on how this phenomenon depends on the AC temperature and on an externally-applied magnetic field. In this scenario, the expertise of our group on strain-tunable QDs is extremely important, as it allows us to match the energy of the single photons emitted by the QD to a standard frequency given by the hyperfine atomic resonance of 87Rb AC. Finally, the outstanding goal would be the implementation of the 87Rb AC as a quantum memory able to store flying qubits emitted by the QDs. As a matter of fact, nowadays the proper functioning of AC quantum memories at room temperature has been demonstrated only for qubits generated by an attenuated laser [4]. This experiment will be performed at the KTH in Stockholm as soon as possible, and probably it will be the first demonstration of quantum memory for photons emitted by QDs.
Another challenge that is often encountered in the realization of a QD-based quantum network is the possibility to interfere single photons emitted by different QDs. So far, experiments have shown visibility of two-photon interference of the order of 50%, a value which is much smaller than the one usually observed in experiments which involve photons emitted by the same QD [7], and in any case lower than the values required to successfully implement quantum gates in quantum networks. The low values of visibility observed in experiments performed by remote QDs is usually ascribed to the fact that QD photons are not Fourier-limited, a hurdle arising from spectral diffusion [8], and phonon-induced decoherence. In this scenario, the development of a FADOF filter will be extremely useful. Indeed, it will allow for finely selecting only those photons whose energy is in a very narrow band, of the order of
hundred MHz. This filter will thus improve visibilities of two-photon interference and, simultaneously, will provide an absolute energy standard in experiments performed with remote QDs. Finally, it is worth noticing that increasing the visibility of two-photon interference is also the key to implement advanced quantum optics experiments as entanglement swapping. While these experiments have been performed with probabilistic single photon sources, such as parametric down conversion, they still must be demonstrated with ondemand quantum emitters.
[1] Chaneliere T., et al., Nature 438, 833 (2005).
[2] Gao W. B., et al., Nature 491, 426 (2012).
[3] Namazi M., et al., Physical Review Applied 8, 034023 (2017).
[4] Wolters J., et al., Physical Review Letters 119, 060502 (2017).
[5] Somaschi N., et al. Nature Photonics 10, 340 (2016).
[6] Vural H., et al., Optica 5, 367 (2018).
[7] Reindl M., ..., and Trotta R., Nano Letters 17, 4090 (2017).
[8] Thoma A., et al., Physical Review Letters 116, 033601 (2016).