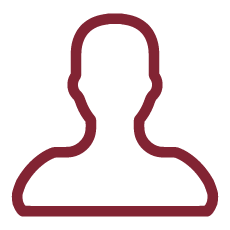
This activity stems from recent achievements of the team of our Department (DIET) on the possibility to obtain nanometric islands of a crystallographic exotic phase of silicon, called Si-III or Si-BC8, diluted in the Si-I or diamond phase of silicon. Si-BC8 phase is mainly appealing because of its electrical and optical features. Indeed, the bulk Si-BC8 phase, obtained in the past mechanically by anvil press on diamond silicon, is known to be a semiconductor with a direct and narrow bandgap (30 meV). The innovative aspect of our method is in obtaining the Si-BC8 phase at low temperature, with a non-destructive planar process fully compatible with the scalability requirements for microelectronics. All these characteristics make our synthesized material amenable for optoelectronic integrated circuit applications in the infrared range of Terahertz (THz). The present one-year project has two main goals: 1) evaluate the possibility to induce Si-BC8 formation with alternative metal species; 2) design and fabricate a THz detector proof of concept. As far as the first goal is concerned, let us mention that, to date, we obtained the Si-BC8 in a microwave-CVD chamber at the Centro per le Nanotecnologie applicatate all¿Ingegneria della Sapienza (CNIS) using Sn as catalyzer metal: in this project we aim to evaluate alternative metal species to Sn for the Si-BC8 synthesis. In the second goal, we plan to pattern the metal spatial distribution on the silicon wafer surface by photolithographic techniques (performed at the DIET clean room) in order to control the Si-BC8 one. The opportunity to control Si-BC8 spatial distribution could pave the way for a THZ detector proof of concept fabrication. The technology steps will be carried out at the DIET and at the CNIS. The optical measurements will be performed at the CNR laboratory in Pisa, where the team has fruitful scientific contacts.
Si-BC8 is regarded as a most promising semiconductor materials for electronics. First, it is amenable to be integrated in the existing silicon IC (Integrated Circuit) manufacturing process. Second it has been found to possess a narrow direct bandgap around 30 meV at room temperature. Therefore, Si-BC8 is suitable for optoelectronic applications in the far IR region (cut-off at 7.25 THz).
The present project takes advantage of a proprietary microwaves-based technique allowing Si-BC8 to be produced without using high energy/high pressure procedures, thus enabling Si-BC8 to be formed directly into Si (diamond) wafers.
Although countless optoelectronic devices have been designed and fabricated over the last 50 years, in the THz frequency range solid-state photodetectors are lacking due to unavailability of semiconductors with high detective quantum efficiency (see Fig.2). For many years astronomers and astrophysicists have taken into consideration gallium doped germanium (Ge:Ga) photoconductors to make observations in the wavelength band above 50 µm [21]. For wavelengths longer than 120 µm stressed Ge:Ga detectors have been developed [22]. These devices respond at wavelengths up to 200 µm and also demonstrate excellent sensitivity. Unfortunately, they require a strong and a massive mechanical stressing harness to extend the long wavelength turn-on. Finally, antimony-doped germanium photoconductors have been tested [23] without achieving sufficient performance to be realistically considered in practical applications.
Thus, in the frame of its formation in diamond silicon wafers, Si-BC8 intriguing optical properties (i.e. semi-metallic band gap) can represent a significant step forward in the direction of solid-state photodetectors for the far IR band, towards the replacement of pyroelectric detectors, thus overcoming the intrinsic pyroelectric selectivity limitations. Besides, Si-BC8 can also be explored as light emission source radiating in the far IR. In addition, such a combination of photosensitive and light emitting elements based on a single, CMOS-compatible material can pave the way to all silicon-monolithic photonic circuits development.
[1] Wentorf, R. H. et al. Science 1963, 139, 338.
[2] Jamienson, J. C. Science 1963, 139, 762.
[3] Hu, J. Z.et al. Phys. Rev. B. 1986, 34 (7), 4679.
[4] Zhang, H. et al. Phys. Rev. Lett. 2017, 118 (April), 14661.
[5] Fadaly, E. M. T. et al. Nature 2020, 580 (April), 205.
[6] Kurakevych, O. O. et al. Inorg. Chem. 2016, 55 (17), 8943¿8950.
[7] Domnich, V. et al. Rev. Adv. Mater. Sci. 2008, 17 (1¿2), 33¿41.
[8] Smith, J. M. J. Appl. Phys. 2012, 112 (8), 083518.
[9] Adam, D. Nature 2003, 421 (6923), p. 571-572.
[10] Kitchen, H. J. et al. Chem. Rev. 2014, 114 (2), 1170¿1206.
[11] Vaucher, S. et al. J. Mater. Res. 2008, 23 (1), 170¿175.
[12] Freeman, S. A. et al. Phys. Rev. Lett. 1995, 74 (11), 2042¿2045.
[13] Robb, G. R. et al. PhysChemComm 2002, 5 (c), 135¿137.
[14] Palma, F. US Patent App. 16/648,080 2020.
[15] Karim, A. et al. Mat. Sci. Eng. 2013, 51 (1), 012001.
[16] Rogalski, A. Opto-Electronics Review 2013, 21, 4, 406-426.
[17] Kurakevych, O. O. et al. Results in Physics 2020, 18, 1032712 (1)- 1032712(9).
[18] Joannopoulos, J. D. et al. Phys. Rev. B 1973, 7(6), 2644-2657.
[19] Biswas, R. et al. Phys. Rev. B 1986, 35(18), 9559-9568.
[20] Malone, B. D. et al. Phys. Rev. B 2008, 78(3), 035210 (1) ¿ 035210 (7).
[21] Wang, J.-Q. et al. Appl. Opt. 1986, 25, 22, 4127-4134.
[22] Wang, J.-Q. et al. Appl. Opt. 1987, 26, 22, 4767-4771
[23] Beeman, J. W. et al. Infrared Phys. Technol. 1996, 37, 7, 715-721.